Superconducting magnets are essential components of today’s particle accelerators and colliders. These magnets come in two types: dipole magnets, which bend the particle bunches to increasingly high speeds (approaching the speed of light), and quadrupole magnets, which squeeze and focus these bunches into the smallest possible size.
Researchers from the Accelerator Technology & Applied Physics (ATAP) Division at Berkeley Lab have played a crucial role in fabricating and assembling more powerful quadrupole magnets based on niobium-tin (Nb3Sn) technology. These magnets have been developed as part of the ongoing contribution of the U.S. Accelerator Upgrade Project to the High Luminosity Large Hadron Collider Accelerator Upgrade Project (HL-LHC AUP), which aims to increase the luminosity of the LHC. The first of several new magnets was delivered to CERN in December.
Quadrupole magnets made from Nb3Sn can produce stronger magnetic fields than the niobium-titanium (Nb-Ti) magnets currently used in the LHC. This results in a more focused particle beam with higher luminosity, expanding the capabilities of the LHC. The new magnets were designed, fabricated, assembled, and tested through close collaboration between Berkeley Lab, Fermi National Accelerator Laboratory (Fermilab), Brookhaven National Laboratory (Brookhaven), and the National High Magnetic Field Laboratory at Florida State University.
“This the first time that magnets made from niobium-tin will be used in a particle accelerator, where they will be installed in the interaction regions of the LHC to increase the rate of collisions in the accelerator,” says Paolo Ferracin, a senior scientist and deputy of ATAP’s Superconducting Magnet Program who leads Berkeley Lab’s contribution to the HL-LHC-AUP.
This increased collision rate, he says, will give researchers “a much better chance of observing rare processes and particles, like the Higgs boson.”
In light of his work on the HL-LHC-AUP, Ferracin was recently invited to co-author a chapter entitled “Large-Aperture High-Field Nb3Sn Quadrupole Magnets for HiLumi” for The Future of the Large Hadron Collider: A Super-Accelerator with Multiple Possible Lives (World Scientific, November 2023). The chapter, co-authored with Giorgio Ambrosio, a senior scientist from the Magnet Technology Division at Fermilab, describes the design, fabrication, and assembly of the Nb3Sn quadrupole superconducting magnets for the LHC upgrade.
“We wanted to provide a summary of the story of these magnets—from their conceptual design and fabrication to assembly and testing—within the context of the book, which provides a fascinating and comprehensive overview of the LHC’s past, present, and future,” explains Ferracin.
Challenges of fabricating Nb3Sn magnets
While the superconducting properties of Nb3Sn exceed those of Nb-Ti, fabricating superconducting magnets made from Nb3Sn is “extremely challenging,” says Ferracin.
For instance, the Nb3Sn cables that wrap around the magnet bore and carry the high currents needed to produce the high magnetic field are very brittle and sensitive to strain. “So you have to be extremely careful when working with them as the risk of damage during fabrication is very high, which increases the risk of degrading their superconducting properties and reducing the performance of the magnets.”
Because of this brittleness, he says conventional wire fabrication techniques, such as extrusion, swaging, and drawing, cannot be used after the superconducting wires are formed. Consequently, the niobium and tin components are separated inside the wire, and the superconducting Nb3Sn compound is formed via reactive diffusion processes only after the coil winding step.
“After the cables are formed, they are sent to Fermilab and Brookhaven, where they are transformed into magnet coils, which are returned to Berkeley Lab, assembled into 4.5-meter-long quadrupole magnets, and tested further at Brookhaven,” says Ferracin.
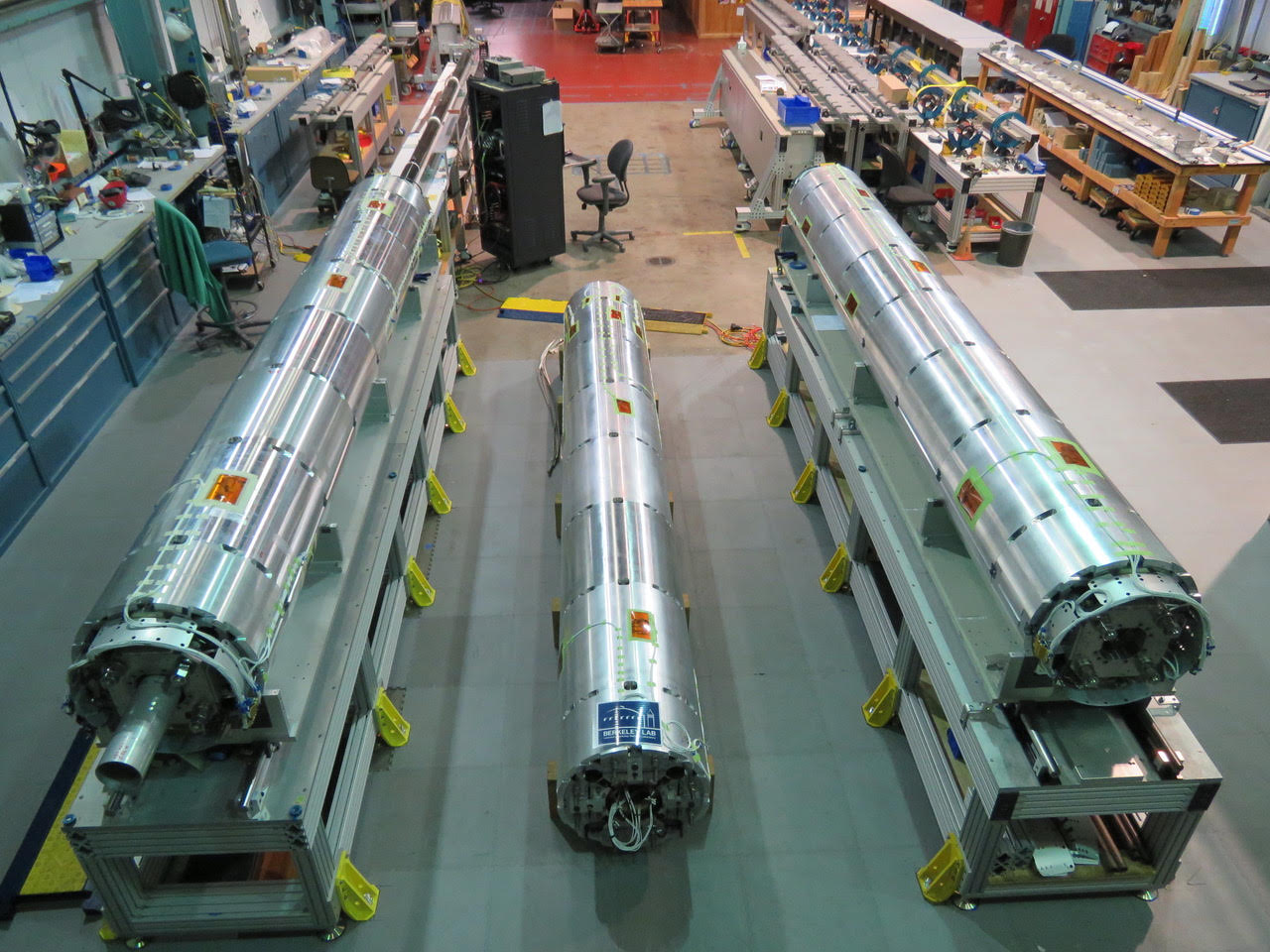
They are then returned to Fermilab, placed in a cryo-assembly, and installed in a stainless-steel pressure vessel (called the Cold Mass), which aligns the magnets in a helium-leak-tight enclosure. After additional testing at Fermilab, this Cold Mass is ready to be shipped to CERN for further testing before installation in the LHC.
Ambrosio noted that these finished magnets “are the outcome of an outstanding collaboration.”
Magnet performance
Before the quadrupole magnets are sent to CERN, they must be tested to ensure they meet the performance requirements stipulated by the HL-LHC-AUP. This involves cooling them to 1.9 K (-456.25 Fahrenheit) to test their operational performance.
“At this temperature, the magnet becomes superconducting, and you need to show that the nominal current can flow through the superconducting cables to produce the desired magnetic field gradient,” explains Ferracin. “The magnet must be able to operate at this nominal current and, therefore, to generate the field gradient set by the project.”
However, he adds, these superconducting cables can experience sudden and unpredictable losses in superconductivity—a phenomenon referred to as quenching—that, if not rapidly detected and controlled, could generate temperatures high enough to destroy the magnets, potentially costing millions of dollars in damage.
Avoiding quenching during operation in the accelerator, says Ferracin, is one of “the most important things we need to achieve.”
To achieve this and ensure optimal performance, the magnets undergo “quench training.” During this process, which is carried out at the Brookhaven test facility, the current in the cables is gradually increased, and the magnets are subjected to all the quenches needed to reach a nominal current; it is then ready to be installed in the accelerator.
“After training, the magnets undergo a thermal cycle, which involves heating them up and then cooling them down again, and a final verification of the nominal current is carried out. This controlled laboratory testing is necessary before the magnets are installed in the hard-to-access subterranean accelerator complex of the LHC,” explains Ferracin.
Fortunately, he adds, that after all this testing, the magnets did not require quenching to reach nominal operational conditions.
“Making physics”
When installed in the LHC, the new quadrupole magnets will produce magnetic fields up to 11-12 tesla—compared with around 8-9 tesla for the existing Nb-Ti magnets.
“With these stronger focusing magnets, the LHC can squeeze the particle bunches into more focused beams (around 10 microns in diameter) at the collision point, significantly increasing the collision rate of the LHC’s proton beams,” says Ferracin.
With this increased rate of collision, he says the LHC will generate up to ten times more collisions in the first 12 years after the upgrade than in the first ten years of the LHC’s lifetime, promising “to extend the frontiers of science and leading to discoveries in particle and high-energy physics and many other areas.”
According to CERN, when operational, the LHC is projected to produce at least 15 million Higgs bosons per year, compared to around three million in 2017.
So far, eight of the planned 20 quadruple magnets, called HL-LHC Inner Triplet quadrupole magnets, or MQXFs, have been developed and tested.
“We have demonstrated that niobium-tin magnets can be used in particle accelerators,” says Ambrosio. “This is great news for high-energy physics, and the lessons we are learning will be extremely valuable for future accelerators.”
The remaining MQXF magnets will be tested at Brookhaven, with testing slated for completion by mid-2025 and installation in the LHC scheduled for completion in 2029, when, says Ferracin, “we start making physics.”
To learn more …
- Major Milestone in Large Hadron Collider Upgrade
- First U.S.-Built Focusing Magnet for LHC Upgrade Arrives at CERN
- Niobium-Tin Superconductors: Fabrication and Applications
- High-Luminosity LHC
For more information on ATAP News articles, contact caw@lbl.gov.